Ever since graphene sheets were isolated from bulk graphite in 2004, two-dimensional materials have received an immense amount of interest for their unexpected and novel electronic and optical properties. These emergent properties have since spurred a rising tide of research into their potential uses in a variety of optoelectronic devices, especially in reducing costs and increasing flexibility of mode-locked lasers.

Graphene hexagonal honeycomb lattice. Hexagonal carbon lattice of graphene, courtesy of Wikimedia commons
Graphene is a two-dimensional material which consists of carbon atoms arranged in a honeycomb lattice structure. In addition to its unique electronic and optical properties, it is relatively cost-effective to fabricate. Its hexagonal lattice structure allows it to be easily integrated into existing electronic and optical devices. The strong in-plane carbon-carbon bonds affords other potential benefits, such as high mechanical strength and flexibility.
Many two-dimensional materials such as graphene and transition metal dichalcogenides (such as MoS2 and WSe2) exhibit strong nonlinear optical effects, which facilitates their use as saturable absorbers in passively mode-locked pulsed laser technology. Mode-locked lasers, or ultrafast lasers, are particularly useful for their ability to deliver high intensity laser pulses on the order of picoseconds or femtoseconds. Compared to continuous laser beams, ultrafast lasers minimize damage to materials being processed or characterized by delivering a high intensity blast of energy over an exceedingly small timespan.
Current State of Saturable Absorption Devices
Saturable absorption is the property of materials whereby the absorption of light decreases with increasing incident light intensity. A saturable absorber therefore acts as an optical switch, where only an optical pulse of sufficient intensity is absorbed by the material and then transmitted as a single laser pulse.
Semiconductor saturable absorber mirrors (SESAMs) have long dominated the market for use in mode-locked lasers, but such devices face limitations such as narrowband operation as well as relatively slow response times, which graphene’s host of unique properties could serve to improve significantly. Whereas SESAMs typically require bandgap engineering and diameter control tailored to the laser output, graphene does not face the same hurdles for a much wider range of light.
Typically saturable absorber materials, such as organic dyes and ion doped crystals face limitations in prohibitively expensive fabrication and implementation. They also tend to have typically narrowband operation. However, graphene absorbs a constant amount of light (typically around 2.3% in the visible to mid infrared light range) at a much larger range of wavelengths. This affords much flexibility in wavelengths of lasers which can be operated using a graphene saturable absorber.
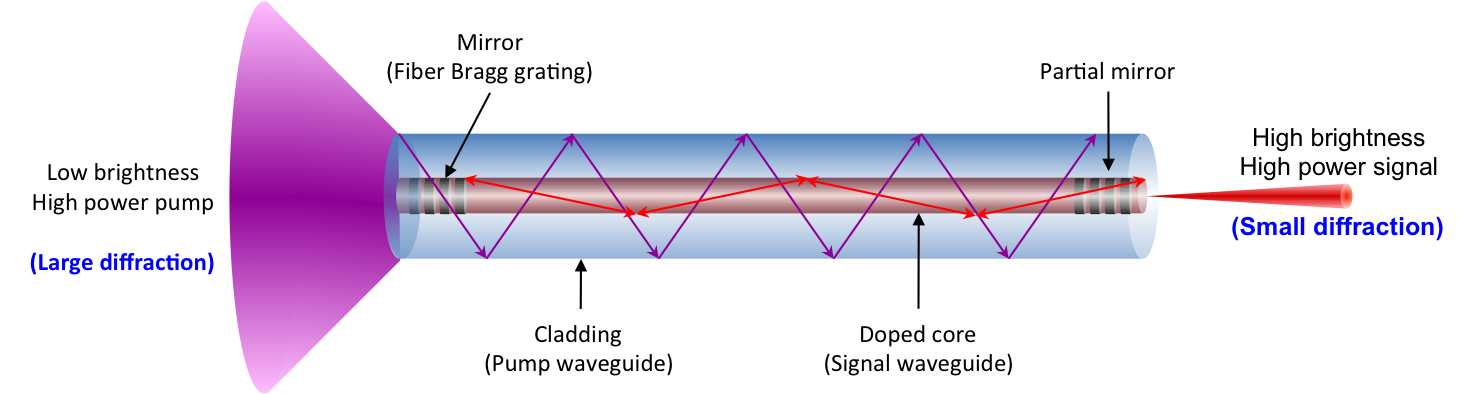
Schematic of high-powered fiber laser. Graphene has demonstrated potential use for its in-plane saturable absorption capabilities when deposited on silicon waveguides in fiber lasers. Image courtesy of Wikimedia Commons
Carbon-based nanostructures have been used for mode-locked lasers before, as many single-wall carbon nanotubes have been demonstrated as effective saturable absorbers as well. However, the intrinsic surface tension present in carbon nanotubes, as well as the inevitable bundling of carbon atoms on the edges leads to a much lower damage threshold, which planar graphene would almost completely circumvent.
Graphene Mode-Locked Lasers
Ultrafast lasers have numerous applications in laser medicine, spectroscopy, and biomedical imaging among other fields. Whereas continuous laser beams can easily damage materials the beam comes into contact with, rapidly pulsed lasers can circumvent this potential hazard. Pulsed lasers suppress heat diffusion into the laser processed material it comes into contact with. This is an important consideration when using laser processing of organic tissues or brittle material. It is especially important is laser manufacturing, where thermal damage of continuous laser beams or long pulse width lasers can cause cracks or other defects that do not appear with rapid laser pulsing.
In laser micromachining, laser pulse directions even on the order of nanoseconds can cause significant swelling and damage to processed areas of metals through melting and heat dissipation. However, femtosecond or picosecond laser pulses bypass such concerns by heating and rapidly vaporizing a relatively small area of affected material.
The precision and high energy intensity of ultrafast lasers is also frequently seen in laser processing of tissue in medicine. Perhaps the most high-profile example of such processing is in LASIK surgery, where high intensity laser incisions necessarily must cause the least possible amount of collateral damage to the cornea.
Ultrafast lasers are frequently used in various medical imaging techniques, specifically multiphoton fluorescence microscopes and fluorescence image guided surgery. Since fluorescence-based imaging requires a nearly monochromatic and intense light source, ultrafast lasers naturally lend themselves to such imaging techniques. Additionally, many biological processes are themselves rapid and require a correspondingly ultrafast spectroscopic methods to capture all information of such processes. Femtosecond lasers are essential technology for analysis of tumors in imaging of cancerous tissue, which you can read more about here.
Future Outlook
Beyond graphene, other 2D materials which exhibit similar nonlinear optical properties have been explored for use in mode-locked lasers. Transition metal dichalcogenides such as MoS2 and WSe2 act as ultrathin semiconductors which exhibit saturable absorption and broadband operation and tunability. Lasers operating in the 1-1.5 micron wavelength regime have been reported with pulse durations in the hundreds of femtoseconds using these materials as saturable absorbers. Other ultrathin materials, such as black phosphorous and bismuth telluride, share similar ease of fabrication and strong nonlinear optical properties.
You can read more about ultrafast fiber lasers, their applications and operating principles on our blog.