Black holes have always fascinated scientists and the public alike. These mysterious objects, with their immense gravitational pull, were once thought to trap everything, including light. But in 1974, Stephen Hawking revolutionized this view with a groundbreaking discovery: black holes can emit radiation. This phenomenon, now known as Hawking radiation, opened new doors in our understanding of quantum mechanics, general relativity, and the fate of black holes. In this article, we dive into the intricacies of Hawking radiation, exploring how it challenges the nature of black holes and reshapes our understanding of the cosmos.
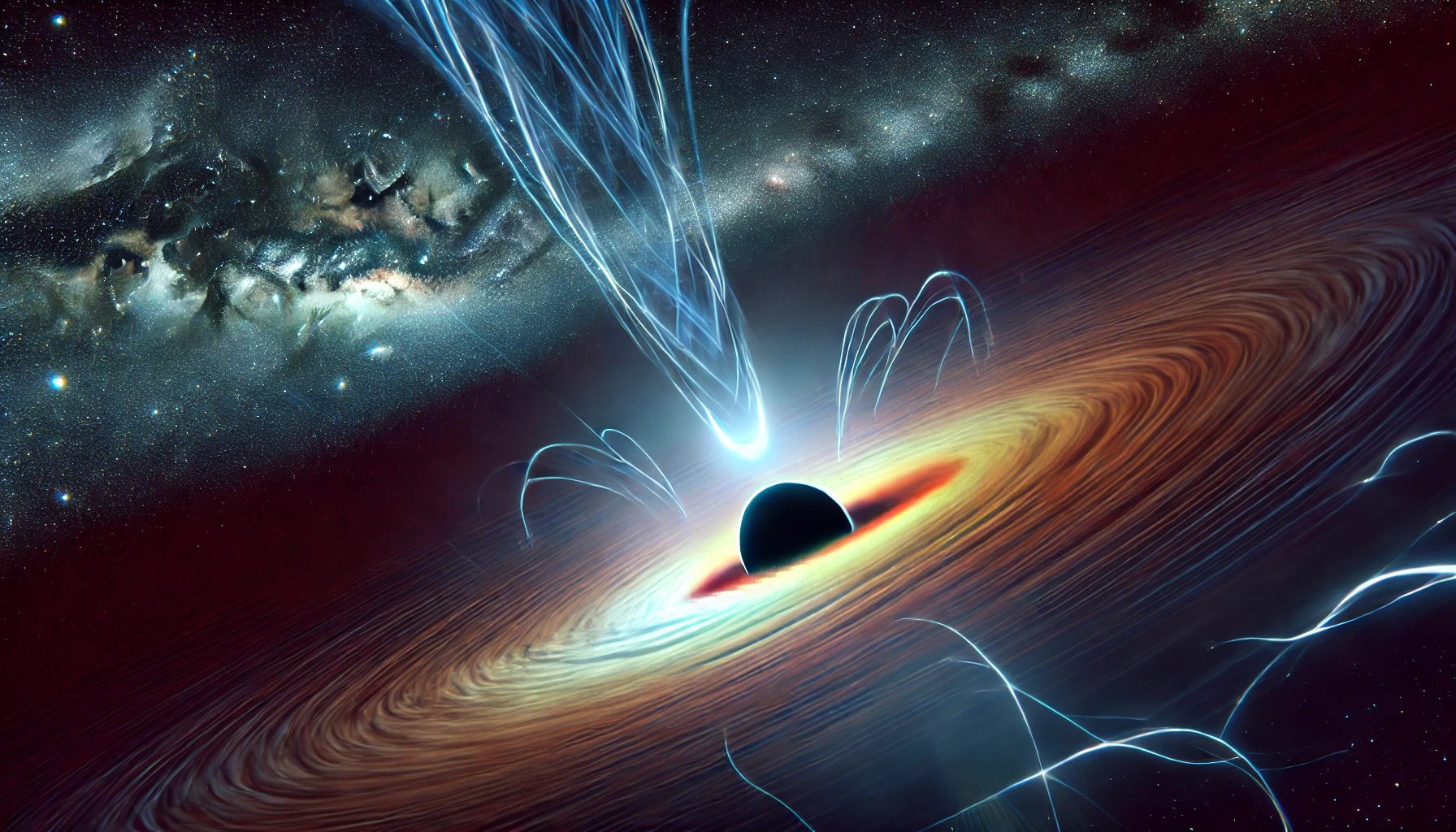
An artistic representation of Hawking radiation, showing faint streams of energy escaping a black hole, symbolizing the slow evaporation of black holes over time.
1. Introduction
Stephen Hawking, one of the most influential theoretical physicists of the 20th century, transformed our understanding of black holes with his groundbreaking work. In 1974, he made an astonishing discovery: black holes, long thought to be cosmic traps from which nothing could escape, are not entirely black. Instead, they emit a faint radiation, now known as Hawking radiation. This revolutionary idea reshaped the way scientists think about black holes, quantum mechanics, and the fabric of the universe itself.
Hawking radiation is significant because it merges two seemingly incompatible pillars of physics—quantum mechanicsand general relativity—into one theory. It provides a window into understanding what happens in the extreme environments surrounding black holes, where gravity is so intense that not even light can escape. Hawking’s work suggests that black holes are not eternal; over time, they can lose mass and eventually vanish entirely, a revelation that adds layers of mystery to their already enigmatic nature.
This discovery has left an indelible mark on our understanding of the cosmos. It forces us to rethink the life cycle of black holes and opens new avenues for exploring the deepest questions in physics, such as the nature of spacetime and the behavior of the universe at its most fundamental level. By bridging the gap between quantum theory and gravity, Hawking radiation not only challenges conventional wisdom but also offers a glimpse into the uncharted territories of science.
2. What is Hawking Radiation?
Hawking radiation is a interesting concept that emerges from the intersection of quantum mechanics and the intense gravitational forces of black holes. In simple terms, it’s the idea that black holes are not entirely black; they emit a small amount of energy in the form of radiation, slowly losing mass over time.
To understand how this happens, imagine the black hole’s event horizon—the boundary beyond which nothing, not even light, can escape. In the vacuum of space, pairs of particles and antiparticles are constantly popping in and out of existence. Normally, these pairs annihilate each other almost immediately. But near the event horizon, something different happens. Due to the immense gravitational pull of the black hole, one of these particles gets pulled into the black hole while the other escapes into space. The particle that escapes carries energy away from the black hole, giving the appearance that the black hole is radiating energy.
This process leads to the black hole gradually losing mass, a phenomenon known as black hole evaporation. Over time, as more and more radiation escapes, the black hole shrinks until it eventually disappears.
What makes Hawking radiation truly remarkable is that it bridges two major fields of physics: quantum mechanics, which governs the behavior of particles at very small scales, and general relativity, which describes how gravity works on large scales, including black holes. Typically, these two theories don’t work well together, but Hawking’s discovery shows how quantum effects can manifest in the extreme gravitational environments around black holes. This revelation hints at deeper, unresolved questions about how these fundamental forces interact and what happens at the boundaries of our current understanding of physics.
3. The Physics Behind It
The physics behind Hawking radiation is rooted in quantum mechanics and the unique environment near a black hole’s event horizon. In empty space, quantum fluctuations constantly create pairs of particles and antiparticles, which typically annihilate each other almost instantly. However, near the event horizon, the extreme gravitational forces of the black hole can separate these pairs before they meet their fate.
When this happens, one particle falls into the black hole while the other escapes into space. The particle that escapes becomes real, carrying energy away from the black hole. From an external observer’s point of view, it looks as though the black hole is emitting radiation. Meanwhile, the particle that falls into the black hole effectively reduces the black hole’s total mass. This process occurs continuously, leading to the slow loss of mass, or black hole evaporation.
This phenomenon ties directly into the thermodynamics of black holes. Just like any physical system, black holes are governed by the laws of thermodynamics. The radiation they emit corresponds to a temperature that depends on their size—the smaller the black hole, the higher its temperature and rate of energy loss. Over time, this gradual energy loss causes the black hole to shrink. Eventually, the black hole could evaporate entirely, leaving behind only radiation.
The combination of quantum mechanics and thermodynamics in this way shows that black holes are not eternal but follow predictable physical laws, transforming our understanding of their life cycles.
4. Why Does it Matter?
Hawking radiation has far-reaching implications for our understanding of black holes and the universe. Traditionally, black holes were thought to be inescapable traps from which nothing—not even light—could return. Hawking’s discovery challenged this notion, showing that black holes are not completely sealed off from the rest of the universe. Instead, they emit energy, losing mass in the process, which suggests they are dynamic objects rather than eternal.
The concept of black hole evaporation fundamentally changes how we view their life cycle. Over time, as Hawking radiation steadily draws energy from a black hole, it begins to shrink. While this process is incredibly slow for large black holes, smaller black holes could evaporate more quickly. Eventually, if left undisturbed, a black hole could completely evaporate, leaving only faint radiation behind.
This evaporation has profound consequences for the ultimate fate of black holes and the universe. If black holes can lose all their mass and disappear, it raises new questions about the nature of matter, energy, and information within them. It also challenges some ideas about the information paradox, which deals with whether the information that falls into a black hole is permanently lost or somehow preserved.
Hawking radiation reshapes the way we think about the cosmos, introducing new ideas about the lifecycle of black holes and pushing us toward a deeper understanding of quantum gravity — the elusive theory that will one day reconcile the laws of the very large (general relativity) with the laws of the very small (quantum mechanics).
5. Debates and Challenges
Hawking radiation, while groundbreaking, has sparked ongoing debates in theoretical physics. One of the biggest challenges is that it highlights a deep conflict between quantum mechanics and general relativity, the two pillars of modern physics. Quantum mechanics governs the behavior of particles at microscopic scales, while general relativity describes gravity and spacetime on cosmic scales. Hawking radiation shows how quantum effects influence black holes, but the two theories remain fundamentally incompatible when applied together at extreme conditions, like near a black hole’s event horizon.
Physicists are working to develop a unified theory, often referred to as quantum gravity, that could reconcile these contradictions. Such a theory would explain how gravity behaves at both large and small scales, shedding light on what happens inside black holes and perhaps even the origins of the universe. However, this goal remains elusive, and Hawking radiation serves as one of the most intriguing pieces of this puzzle.
A particularly pressing question is the information paradox. According to quantum mechanics, information about the state of a system cannot be destroyed. But if a black hole eventually evaporates due to Hawking radiation, what happens to the information about the objects that fell into it? Is the information lost forever, or is it somehow encoded in the radiation that escapes? This paradox has led to heated debates, with some physicists proposing that information is preserved in subtle quantum states, while others suggest that it may truly be lost.
These challenges keep Hawking radiation at the center of some of the most fundamental questions in physics, pushing the boundaries of our understanding of the universe.
6. Potential Observations and Experimental Evidence
One of the greatest challenges in studying Hawking radiation is that it emits an extremely weak signal, making it nearly impossible to observe directly. For large black holes, the radiation is so faint that it’s overshadowed by cosmic background radiation. Only for very small black holes, which would emit higher levels of radiation, might we have a chance of detecting it. However, no such tiny black holes are known to exist nearby, and creating them in laboratory conditions is far beyond our current technological capabilities.
Despite these obstacles, physicists have been working on theoretical and experimental advances to provide indirect evidence of Hawking radiation. Some researchers are using analog systems—such as sonic black holes or other experimental setups that mimic the behavior of black holes through fluid dynamics or laser physics. These systems simulate event horizons, where similar particle-pair behavior can be observed. While these setups are not black holes, they provide valuable insights and offer an indirect glimpse into how Hawking radiation might behave.
Additionally, there are theoretical advancements involving quantum field theory and string theory, which suggest that Hawking radiation could be tied to more general quantum processes. As we continue to improve our understanding of quantum mechanics and gravity, new approaches may one day lead to more concrete observational evidence, even if direct detection remains out of reach for now.
These experimental and theoretical efforts, though still in their early stages, represent humanity’s persistent quest to verify one of the most fascinating predictions in modern physics.
7. Conclusion
Hawking radiation stands as one of the most remarkable discoveries in modern physics, offering a glimpse into the deep and mysterious workings of black holes. By revealing that black holes emit energy and slowly evaporate over time, this theory has reshaped our understanding of these enigmatic objects and challenged the traditional view that they are inescapable. Its significance goes beyond black holes, bridging the gap between quantum mechanics and general relativity, and raising profound questions about the nature of space, time, and the universe.
Beyond its theoretical importance, Hawking radiation opens new doors for exploring the intersection of the largest and smallest forces in the cosmos. It pushes us closer to finding a unified theory of quantum gravity, a holy grail in theoretical physics. And while direct observation remains a distant goal due to its incredibly weak signal, the ongoing efforts to understand and verify it continue to inspire breakthroughs across multiple fields.
As we probe deeper into the mysteries of the universe, one question remains: Will we ever directly observe Hawking radiation and unlock the full secrets of black holes? The answer may lead us to new realms of discovery, transforming our understanding of the cosmos forever.