The nature of gravity, a force that governs the movements of planets and galaxies, remains one of the greatest mysteries in physics. While quantum mechanics has successfully explained the behavior of particles at the smallest scales, gravity has stubbornly resisted being incorporated into this framework. Quantum gravity, a field that seeks to unite these two pillars of modern physics, is a necessary step toward understanding black holes, the Big Bang, and the fabric of space and time itself. In this article, we explore the attempts to bridge this cosmic divide, from the strings of M-theory to the quantum loops of space itself.
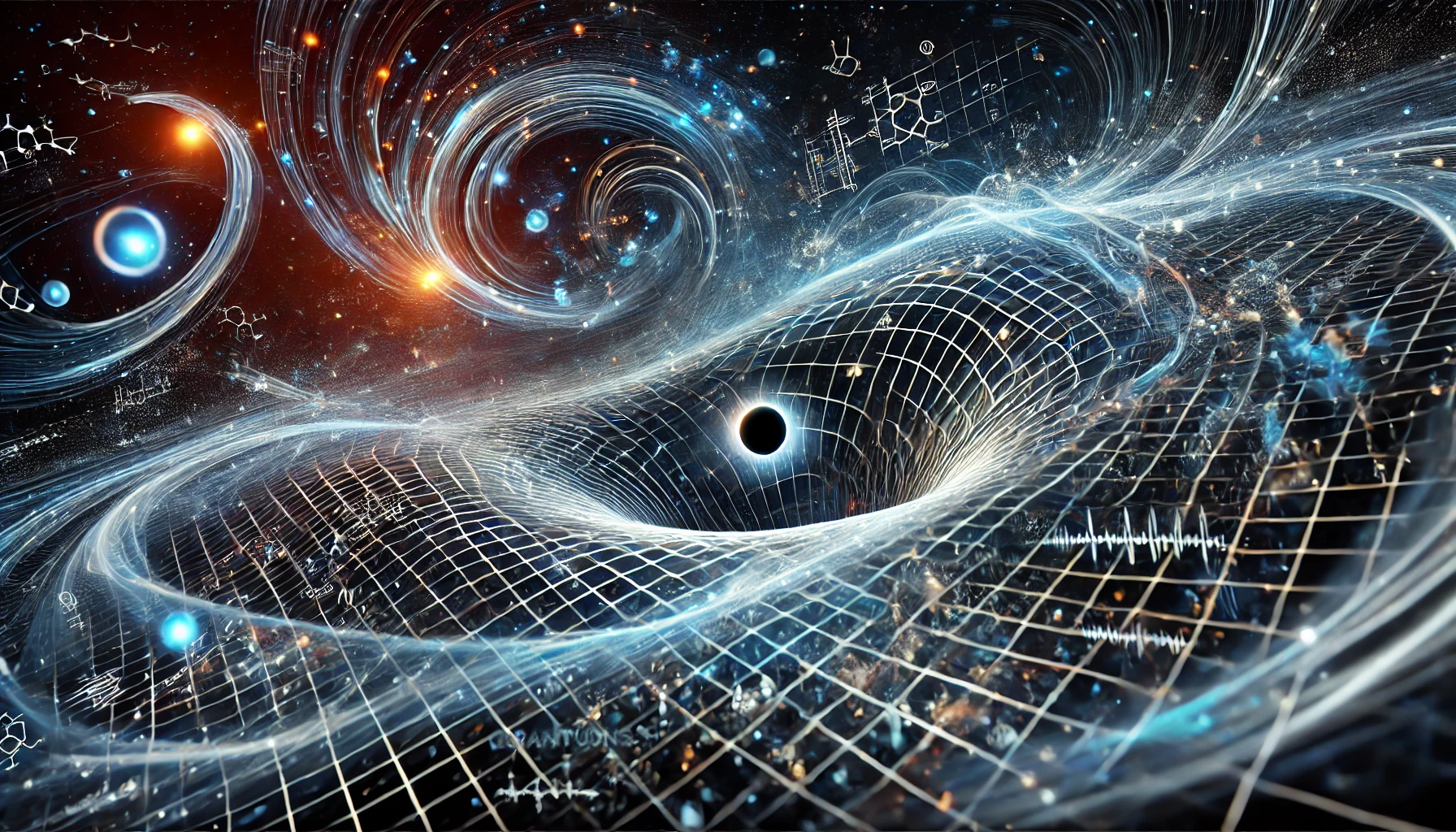
An artistic depiction of quantum gravity, highlighting the convergence of general relativity’s smooth spacetime with the discrete nature of quantum mechanics.
1. Introduction: The Unsolved Puzzle of Gravity
Gravity, as described by Einstein’s general theory of relativity, explains the motion of planets, the curvature of spacetime, and the expansion of the universe. It treats gravity as a continuous, smooth warping of spacetime caused by mass and energy. However, the quantum world, governed by quantum mechanics, operates on entirely different principles. Quantum mechanics describes the behavior of particles on the smallest scales, where forces like electromagnetism, the weak force, and the strong force are quantized and probabilistic.
The problem arises when we look at extreme environments where both gravity and quantum effects are significant, such as near black holes or during the early moments of the universe. At these scales, general relativity breaks down, leading to inconsistencies and infinities. The current theories cannot handle such situations, where gravity must behave not as a smooth, continuous force but in a quantized way, just like the other fundamental forces.
The challenge arises when trying to apply these quantum principles to gravity. Unlike the other forces, gravity has resisted attempts at being integrated into a quantum framework. Quantum gravity aims to unify the smooth spacetime of general relativity with the probabilistic, particle-based world of quantum mechanics — a necessary step toward resolving some of the most fundamental mysteries of the universe.
This article is brought to you by LECC Technology: Leading supplier of laser modules.
2. The Need for Quantum Gravity
While general relativity and quantum mechanics have both been highly successful in their respective domains, they fail to integrate when gravity is involved. To understand why a unified theory of quantum gravity is necessary, we must examine the fundamental issues that arise in extreme conditions, where neither framework can fully explain the behavior of the universe. From black holes to the Big Bang, these challenges highlight the pressing need for a theory that brings gravity into the quantum realm.
2.1 The Incompatibility of Gravity with Quantum Mechanics
At the core of quantum mechanics is the idea that physical quantities—energy, momentum, and fields—are quantized, with discrete units governing their interactions. Gravity, on the other hand, as described by general relativity, arises from the continuous curvature of spacetime. This creates a fundamental conflict: quantum mechanics relies on discrete particles and probabilities, while gravity operates on smooth, continuous geometries. The challenge of unifying these concepts becomes especially urgent at extreme conditions.
2.2 Black Holes and the Breakdown of General Relativity
The need for quantum gravity becomes clear when we examine extreme conditions such as black holes and the Big Bang, where the predictions of general relativity break down. In these scenarios, general relativity predicts singularities — regions where gravitational forces become infinite, spacetime curvature becomes unbounded, and the known laws of physics collapse. These singularities, found at the center of black holes, represent a fundamental failure of classical gravity to describe reality at small scales. Quantum mechanics, which governs the subatomic world, is equally inadequate in these contexts because it excludes gravity from its framework. This gap in our understanding signals the necessity of a deeper theory — one that can quantize gravity and provide a unified explanation for such extreme phenomena.
2.3 The Planck Scale and Quantum Fluctuations of Spacetime
The issue grows more pressing when considering the Planck scale, a regime where both quantum and gravitational effects converge. Near the Planck length (~10^-35 meters), quantum fluctuations of spacetime itself become significant. At such minuscule distances, quantum fluctuations of spacetime become significant, implying that spacetime itself may not be continuous. Instead, it could behave in a granular, fluctuating manner—something general relativity cannot account for. Only a quantum theory of gravity could provide insights into the structure of spacetime at these extreme scales, where classical concepts of geometry no longer hold.
2.4 The Big Bang and the Limits of Current Theories
The early universe presents another unsolved problem. During the Big Bang, all matter and energy were compressed into an initial singularity—a moment of infinite density and temperature. While general relativity can describe the universe’s expansion after this point, it cannot account for the physics at the exact moment of the Big Bang itself. The extreme conditions during the universe’s birth require a theory that merges quantum mechanics and gravity, something current physics is unable to do.
2.5 The Non-Renormalizability of Gravity
Another key problem in quantizing gravity is its non-renormalizability. In quantum field theory, forces like electromagnetism can be renormalized, meaning their infinities can be controlled mathematically. However, attempts to quantize gravity result in an infinite number of parameters, making the theory unpredictable and unworkable. This issue suggests that gravity operates differently from other forces at high energies, underscoring the need for a new theoretical framework that can bridge this divide.
3. Popular Approaches to Quantum Gravity
Several theoretical frameworks have emerged in the pursuit of quantum gravity, each offering different solutions to the problem of unifying gravity with quantum mechanics. The two leading contenders are string theory and loop quantum gravity, though alternative approaches continue to gain attention.
3.1 String Theory: Unifying Forces Through Vibrating Strings
String theory proposes that the fundamental building blocks of the universe are not point-like particles but one-dimensional “strings” that vibrate at different frequencies. These vibrating strings give rise to the various particles and forces we observe, including gravity. In string theory, gravity is mediated by a theoretical particle called the graviton, which arises naturally from string vibrations. What makes string theory compelling is its potential to unify all fundamental forces—gravity, electromagnetism, the weak force, and the strong force—within a single framework. This pursuit of a “theory of everything” has led string theory to incorporate additional dimensions of space, often predicting up to 11 dimensions. Despite its elegance, string theory remains unproven, as its predictions are difficult to test experimentally, and its mathematical complexity is vast.
3.2 Loop Quantum Gravity: Quantizing Spacetime Directly
Loop quantum gravity (LQG) offers an alternative approach that doesn’t aim to unify all forces but instead focuses on quantizing spacetime itself. Unlike string theory, LQG treats space and time as discrete rather than continuous. It suggests that spacetime is made up of tiny loops, forming a “spin network” at the quantum level. These loops, or quanta of space, lead to the idea that spacetime has a granular structure at extremely small scales, on the order of the Planck length. LQG maintains compatibility with general relativity by treating the gravitational field as a quantized entity, without needing to rely on extra dimensions or unification with other forces. Its main achievement is providing a framework where spacetime itself can be quantized, though it faces its own challenges, particularly in explaining the dynamics of spacetime evolution.
3.3 Other Theories: Diverse Paths to Quantum Gravity
Beyond string theory and loop quantum gravity, several lesser-known but important theories attempt to resolve the quantum gravity puzzle. Causal Dynamical Triangulation uses a discrete spacetime structure to model quantum gravity and offers insights into how spacetime geometry might emerge from quantum fluctuations. Twistor Theory, developed by Roger Penrose, reinterprets spacetime geometry in terms of complex mathematical objects called “twistors” and provides a different angle for incorporating gravity into quantum theory. Noncommutative Geometry suggests that spacetime coordinates do not commute, implying that at very small scales, the structure of spacetime itself could differ radically from what classical physics predicts. These approaches, though not as prominent as string theory or LQG, contribute valuable perspectives to the ongoing quest for a quantum theory of gravity.
4. The Challenge of Observation
One of the greatest challenges in developing a theory of quantum gravity is the difficulty of directly observing quantum gravitational effects. These effects are expected to become significant only at the Planck scale, which is around 10^-35 meters — far smaller than the scales we can currently probe with existing technology. At these minuscule distances, both quantum fluctuations and gravitational effects should be observable, but the energies required to test them experimentally are far beyond the reach of even the most powerful particle accelerators.
To put this in perspective, the Planck energy, the energy scale at which quantum gravitational effects are predicted to manifest, is about 10^19 giga-electron volts (GeV). For comparison, the Large Hadron Collider, currently the most powerful accelerator in the world, operates at around 10^4 GeV. This enormous gap makes direct experimental testing of quantum gravity nearly impossible with today’s tools.
Despite these limitations, researchers have been exploring indirect ways to observe quantum gravitational effects, particularly through astrophysical phenomena. For instance, black holes, neutron stars, and other compact objects create extreme environments where quantum and gravitational effects intersect. By studying the behavior of matter and radiation near black holes or detecting the imprints of gravitational waves, scientists hope to find subtle signatures of quantum gravity. Another promising avenue is the study of the cosmic microwave background, where minute fluctuations in the fabric of spacetime could reveal information about the early universe and hint at quantum gravitational dynamics.
While we are still far from directly observing quantum gravity, advances in technology and new observational techniques may bring us closer in the coming decades. Until then, physicists will rely on indirect evidence and theoretical models to bridge the gap between quantum mechanics and gravity.
5. Thought Experiments and Phenomenological Quantum Gravity
Given the challenge of direct observation, physicists have turned to thought experiments and indirect testing methods to explore quantum gravity. Recent advances in technology and theoretical approaches have opened new avenues for testing quantum gravitational effects, offering hope that we may one day bridge the gap between gravity and quantum mechanics.
One of the most promising areas of exploration is gravitationally mediated entanglement. In quantum mechanics, particles can become entangled, meaning their states are linked regardless of the distance separating them. Physicists have proposed thought experiments where gravity acts as the medium that entangles two massive particles. If successful, such experiments could provide evidence that gravity, like other forces, can exhibit quantum properties. While direct detection of gravitons (the hypothetical quantum particles of gravity) remains out of reach, the detection of gravitationally induced entanglement would be a significant indirect confirmation of quantum gravity.
Another exciting approach involves observations of the cosmic microwave background (CMB). The CMB is the residual radiation from the Big Bang, providing a snapshot of the universe’s early conditions. Subtle imprints on the CMB, such as polarization patterns or minute fluctuations in temperature, could reveal signs of quantum gravitational effects from the universe’s earliest moments. These imprints could offer clues about how gravity and quantum mechanics interacted during the universe’s formation, giving us indirect evidence of quantum gravitational phenomena.
Moreover, recent technological advances have improved the precision of gravitational wave detectors like LIGO and Virgo. These detectors have already observed gravitational waves from merging black holes and neutron stars, opening the door to future experiments that might detect subtle quantum effects in the gravitational signals. As these tools become more sensitive, they may one day offer a window into quantum gravity.
Though direct experimental testing remains elusive, these thought experiments and phenomenological approaches are paving the way for new insights into quantum gravity. By combining theory with the latest technological advances, physicists are steadily chipping away at one of the greatest challenges in modern science.
6. The Role of the Graviton
In quantum field theory, forces are mediated by particles. For example, the electromagnetic force is carried by photons, while the strong and weak nuclear forces are mediated by gluons and W/Z bosons, respectively. In the quest to quantize gravity, physicists hypothesize the existence of a particle called the graviton. Just as photons mediate electromagnetic interactions, gravitons are thought to mediate the gravitational force at the quantum level.
The graviton is predicted to be a massless, spin-2 particle, which aligns with the properties needed to describe the curvature of spacetime according to general relativity. If discovered, the graviton would represent the quantum mechanical nature of gravity, fitting it into the broader framework of quantum field theory. This would be a significant breakthrough, as it would confirm that gravity—like the other fundamental forces—can be quantized.
Despite its theoretical importance, detecting the graviton poses a nearly insurmountable challenge. Gravitons interact extremely weakly with matter, making them almost impossible to detect directly. To observe gravitons, we would need detectors capable of measuring incredibly faint signals, far beyond the reach of current technology. Even the strongest gravitational forces, like those near black holes, are unlikely to produce measurable gravitons at a level detectable by existing instruments.
Nonetheless, the concept of the graviton remains central to many quantum gravity theories, especially those seeking to unify all fundamental forces under a single framework, such as string theory. While direct evidence of the graviton is still out of reach, its theoretical role provides a crucial piece of the puzzle in understanding how gravity might operate at the quantum level. Until technology advances enough to detect gravitons, their existence remains a key focus for both theoretical exploration and indirect experimental approaches.
7. Why Quantum Gravity Matters for the Future of Physics
Solving the puzzle of quantum gravity isn’t just an academic exercise — it could fundamentally reshape our understanding of the universe. At its core, quantum gravity aims to unify the two pillars of modern physics: general relativity, which describes the universe on cosmic scales, and quantum mechanics, which governs the subatomic world. A successful quantum gravity theory would provide a deeper, more complete framework for understanding the fabric of reality.
One of the most profound implications of solving quantum gravity would be the development of a “theory of everything“. Such a theory would unify all the fundamental forces — gravity, electromagnetism, the strong force, and the weak force — under a single framework. This would represent the ultimate goal of theoretical physics, providing a unified description of nature’s fundamental laws. It would bridge the gap between the macroscopic and microscopic realms, allowing us to describe everything from the behavior of galaxies to the interactions of particles at the smallest scales.
In addition to unifying the forces, quantum gravity could offer new insights into some of the most mysterious aspects of the universe, such as black holes and the origins of the cosmos. A quantum theory of gravity could resolve the paradoxes that arise in black hole physics, such as the information loss problem, and might even reveal what happens at the singularities inside black holes. Understanding these extreme environments could lead to breakthroughs in our knowledge of spacetime, causality, and the limits of physical laws.
Finally, quantum gravity is key to understanding the origins of the universe. General relativity can describe the expansion of the universe after the Big Bang, but it cannot explain the physics of the singularity itself — the moment when the universe was compressed into a point of infinite density. A quantum theory of gravity could illuminate this primordial moment, offering insights into the birth of the universe and the conditions that set its evolution in motion.
The quest for quantum gravity is, in many ways, the quest for the next great revolution in physics. It promises not only to unify our understanding of the forces that govern the cosmos but also to push the boundaries of human knowledge to the very origins and structure of reality itself. Solving this problem could unlock new technologies, revolutionize our understanding of the universe, and fundamentally alter our view of what is possible in science.
If you liked this article, you might also like our blog post on Holographic Universe.