Stimulated emission depletion microscopy (STED) is a popular super-resolution microscopy technique that researchers use to magnify microscopic organic compounds and proteins. This method is superior to conventional light microscopy as the images have less noise and are sharper given that the inspected molecule is a living organism. This is because STED microscopy leaps over the issues revolving around the diffraction limit that cripple regular microscopes. STED has solid reliability in the biological field where scientists have to observe living cells that emit fluorescence light. Using both an excitation and depletion laser, researchers can target dimensions smaller than 200 nm within a cell and create a high-resolution image with multiple sample images. In this article, we will briefly go over these topics of STED microscopy.
- Visible Light Microscopy Coming To An End?
- How STED Microscopy Works
- Variation of STED Microscopes
- Applications
- Conclusion
Visible Light Microscopy Coming To An End?
Visible light microscopy uses, just as the title suggests, visible light in the 400-700nm range. The performance limit of such microscopes is determined by the diffraction limited performance of the lenses used in the microscope. Using shorter wavelength of light and a larger numerical aperture of the lens, a diffraction limited performance can be achieved for visible microscopes. Modern equipment can reach a numerical aperture of roughly 1.4 using immersion technology (where the medium between the objective lens and the sample is immersed in fluid medium with refractive index higher than that of air), leading to a lateral resolution of around 250nm. Anything smaller causes image blur. As shown on Figure 1, the Abe equation for the minimum resolvable distance between features is given by the average wavelength over the quantity of two times n (refractive index) and sin(acceptance angle or angle of view). n*Sin(a) is the numerical aperture (N.A.) of the objective/lens. This relationship is also known as the Abbe’s diffraction limit. STED microscopy allows bypassing the diffraction limit by creating super-resolution images by the selective deactivation of fluorophores. This is achieved by minimizing the focal point illuminated by the excitation light, and thus enhancing the achievable resolution.
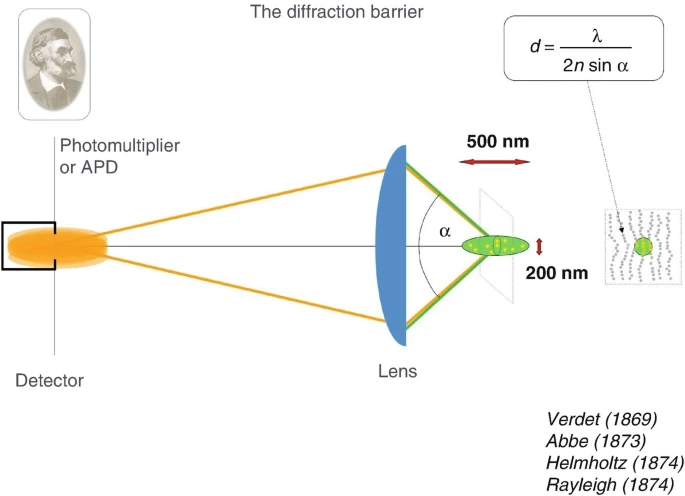
How confocal microscopy works and the diffraction barrier equation associated with it. Image sourced from the article: High-Resolution 3D Light Microscopy with STED and RESOLFT.
How STED Microscopy Works
STED microscopy utilizes the absorption and emission of fluorescent dyes. A laser is pointed at certain areas of interest where it will emit two different wavelengths. One wavelength at the level where the sample will absorb the energy and reemit it as fluorescent light. A secondary laser, called a depletion laser, is then activated shortly after the first beam; this wavelength is red-shifted compared to the primary laser and will create a donut around the first beam. This second beam will turn “off” the molecules in the region, allowing the detector to capture only the region of the first excitation, where the molecule will be glowing due to the fluorescent light. This allows the microscope to see the finest of details. These lasers are switched in picosecond time frames, capturing areas with extreme precision. The donut shape of the second beam can be adjusted in size to accommodate the size of the sample that is being absorbed. Scanning a large area with successive beams will result in a large image once all the sections are captured and stitched together.
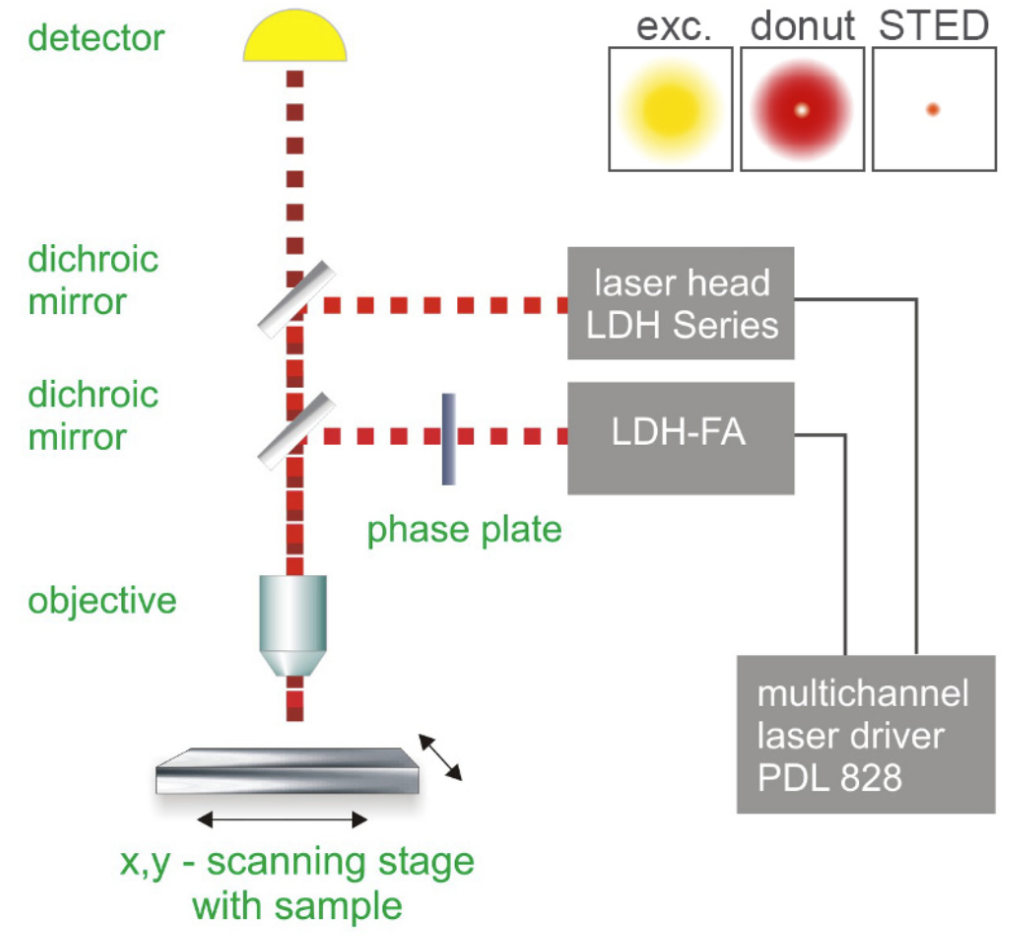
How STED microscopy works. Image courtesy of PicoQuant
Variations of STED Microscopy
Pulsed STED
Pulsed STED is where the excitation and redshifted light are emitted right after one another, this is how STED was first introduced. Pulsing these lasers in multiple random regions will create a high-resolution image of the sample that is being examined. These pulses can either be activated in rows or can be randomized, normally being activated for only 100 picoseconds or less. Moving from row to row may result in molecules remaining excited from the previous zap, resulting in a noisy image. However, this can be fixed by having an excitation pulse emitted just onto the desired molecule. Then around 300 picoseconds later, the depletion pulse is activated for a longer period of time, silencing the region. This ensures that the molecule is completely depleted of any fluorescence light and the laser can move to the next target. The article outlining STED states that the excitation pulse must be much shorter than the STED depletion pulse to decrease the chances of noisy data.
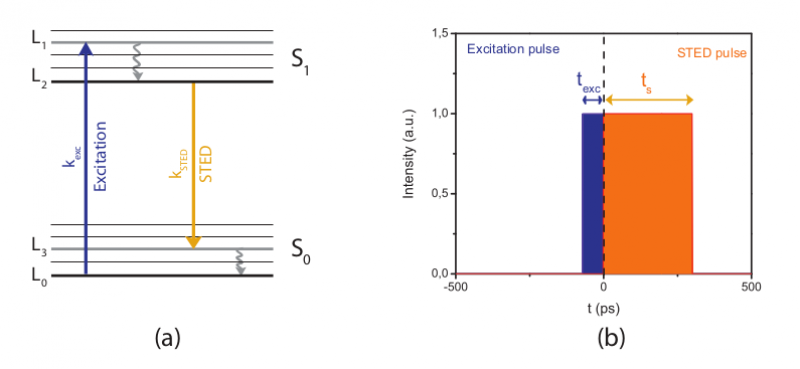
Gated Regions of STED microscopy. Image courtesy of Science Volume Imaging.
Continuous Wave (CW) STED
In CW STED, both the excitation and depletion laser will be simultaneously on, which results in scans that do not have to rely on single pulses to create an entire image. Since the microscope can scan entire rows at a time, this can be an efficient method as the pulsed STED can be time-consuming for larger images. However, CW STED can result in some molecules being still luminous after the laser has passed the area. This stray fluorescent light can cause noise to the image, decreasing the quality. Therefore, this method isn’t widely used to develop high-resolution scans.
Gated STED
Combining properties of both pulsed and CW STED methods, gated STED allows us to maximize the benefits of the two approaches to STED. Since CW STED microscopy is noisy due to the depletion beam not eliminating the entire excited region, gated STED turns the excitation beam on for a brief amount of time. This mechanism takes advantage of the time that it takes for the fluorophores to completely diminish. Doing a simple experiment to find this time, researchers can assign the microscope to turn off the excitation beam for the calculated amount of time. This will reduce the extraneous fluorescent light being emitted to the detector.
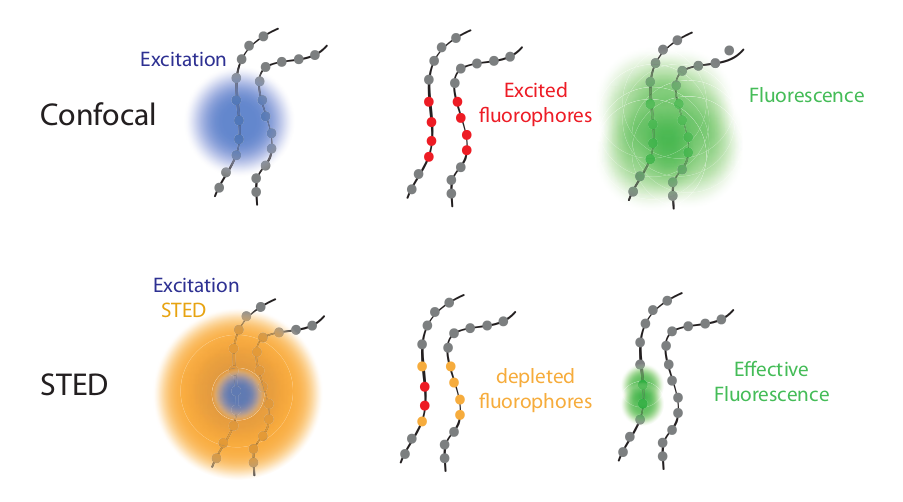
How STED microscopy utilizes fluorescent absorption and emission. Image courtesy of Science Volume Imaging.
Applications of STED Microscopy
As mentioned above, STED has made remarkable progress in the past two decades, most notably in the biomedical field. It has allowed researchers to understand protein interactions, which occur on a scale of a few nanometers. Since the diffraction limit is around 200nm to 250nm, an optical method would not be sufficient for visualizing these subtle events. STED enables scientists to observe these interactions below 50 nm. Most of the time, fluorescent beads are used to determine the magnification level of microscopes. STED excels in these tests as fluorescent lighting is one of its strongest features. With enhanced features, scientists could see cell interactions within bodies. They were able to pick out features of metallic particles and the interplay between cancer cells. Additionally, the study of nanoparticles can be further researched as cells can be viewed in higher resolution. These nanoparticles can either disrupt or enhance mechanisms that can be visible with fluorescent tags.
STED microscopy has also allowed viewing living cell interactions. For example, fluorescent labeled cholesterol was fed to cells and tested using spectroscopy and STED microscopy. Researchers were able to discover how reliant cells are on cholesterol and actin polymerization. Given that a range of florescent labeling agents can be attached to a select protein, researchers were also able to see the distribution of these strands within the organism and pinpoint exact locations and variations. With these same protein labeling features, scientists used two different excitation wavelengths. In this method, researchers could separate the various species in a living organism. They achieved this by exciting the sample with two wavelengths that were close in energy to separate different organic molecules.
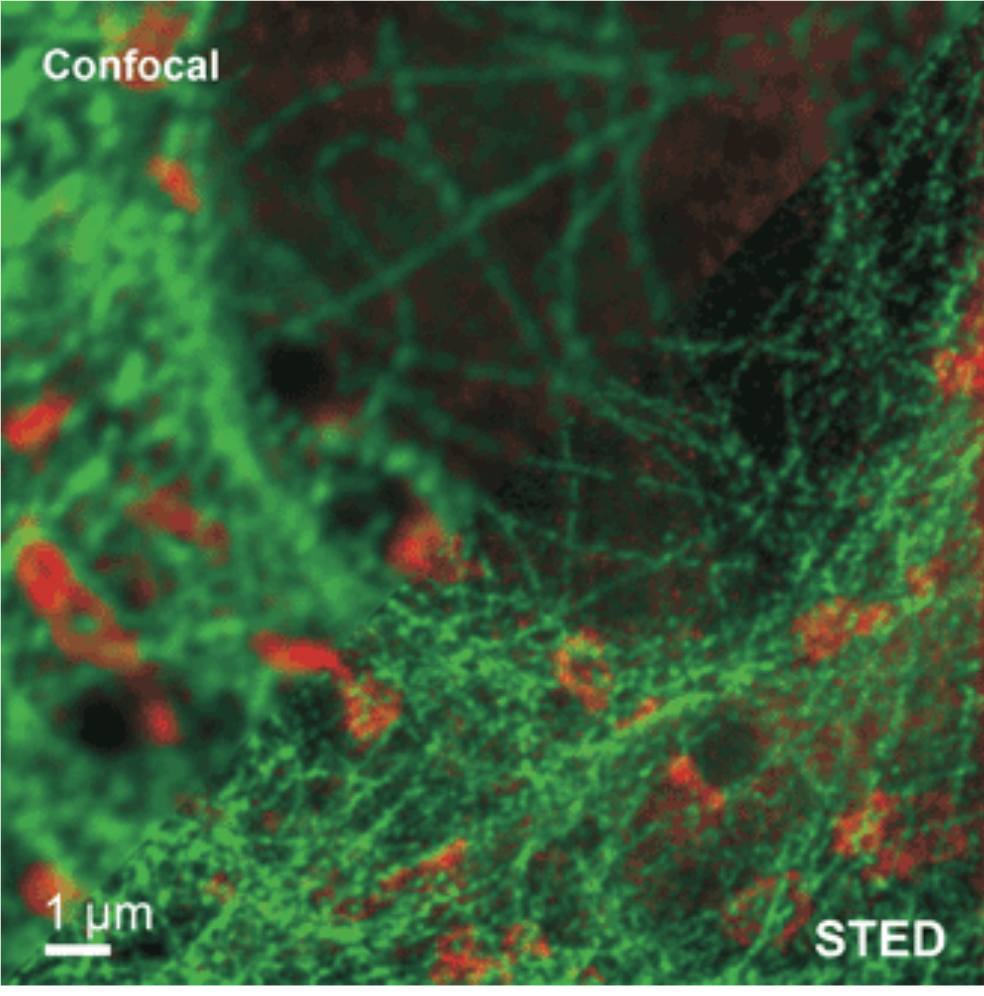
STED detecting two different species in one sample, comparing to confocal methods. Image courtesy of phtonicsmedia.
Conclusion
With STED having two different states, on and off, scientists can create high-resolution images of proteins and living molecules by using stimulated emission. By taking small bits of an image, they can combine it into one large photo with incredible detail that has never been achieved before. The one downside is that this is only effective for specimens that can absorb and reemit fluorescence light. Even though confocal microscopy has been the go-to for decades, STED microscopy has been leading in resolution and is becoming the standard for microscopy in the biomedical field.